Very close to fusion energy: Plasma-facing materials
Ever since Hermann Ludwig Ferdinand von Helmholtz definitively stated the law of conservation of energy in 1847, scientists have been trying to find out the cause of the un’s light. And the first plausible conjectures about this question were meteoric. Formulated by Julius Robert Mayer and John James Waterston, they assumed that heavy meteoroid precipitation fed energy into the Sun. However, these arguments were soon abandoned when it was realised that even such a bombardment could not generate the required energy. Thus, from 1860 onwards, the Helmholtz-Kelvin hypothesis was accepted, which attributed sunlight to a permanent conversion of gravitational energy into heat as the Sun contracted. The idea was accepted with some reluctance, as Kelvin’s calculations on this basis predicted an age for the Sun of no more than 30 million years, in sharp conflict with accepted geological models, the fossil record and Darwin’s evolutionary theory.
The solution to the riddle can be dated back to 1939. That year Hans Albrecht Bethe proved beyond doubt that stars are crucibles where hydrogen nuclei fuse, releasing the formidable energy heralded by Albert Einstein’s celebrated relativistic formula. However, almost two decades earlier, Jean Baptiste Perrin and Arthur Stanley Eddington had already ventured some ideas in this respect [1]. In fact, the latter stated, in 1920 during a lecture in Cardiff, that “a star is drawing on some vast reservoir of energy by means unknown to us. This reservoir can scarcely be other than the subatomic energy which, it is known, exists abundantly in all matter; we sometimes dream that man will one day learn how to release it and use it for his service. The store is well-nigh inexhaustible, if only it could be tapped. There is sufficient in the Sun to maintain its output of heat for 15 billion years”.
It has long been joked that commercially producing electricity by fusion was always fifty years away. Now, many researchers are confident that the fifty-year “constant” has been broken, and our generation, perhaps closer to the tradition of Vesta than Prometheus, will be fortunate enough to see Eddington’s dream come true. For the second time in history mankind will control fire. And this time it is the one that burns in the heart of the stars.
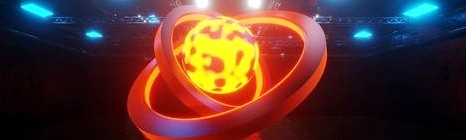
But let’s not kid ourselves: As we have seen in this series of articles, stably bottling plasma under ignition conditions —in terms of time, temperature and density, as John David Lawson taught us in 1957— is still a tricky challenge [1]. And not only that. The operation of a fusion reactor requires that its structural components, particularly its vessel, meet stringent safety standards. These conditions considerably limit the number of materials available. So… what characteristics do these need to have in order to be used in the fusion environment?
[1] Eddington and Perrin’s postulate was supported both by the cohesion coefficient introduced by Francis William Aston (when he found that the fusion of hydrogen into helium, due to the mass defect, released enough energy to satisfy solar demands) and by Henry Norris Russell’s discovery of the Sun’s chemical composition.
[2] In stars, and our Sun is one of them, the fusion process is driven and maintained by its enormous gravitational mass, so to bring this power to Earth we need a different strategy, e.g. heating the fuel gases to temperatures of about 150 MK, seeking to trigger fusion by thermal agitation. At these very high temperatures, the positively charged atomic nuclei are stripped of their orbital electrons. In other words, the gas becomes a plasma. Experts know how to heat a plasma to such temperatures, but there is no material that can hold such prodigiously hot plasmas. This is where the magic of strong magnetic fields comes in, making it possible to “bottle” such hot plasmas.
Inside a fusion reactor
It was already stated elsewhere that the most promising reaction for powering the first generation of commercial fusion power plants is that of deuterium and tritium (D-T), more precisely that of deuteron (2H+) and triton (3H+), because at ignition plasma temperatures above 150 MK, necessary to overcome the Coulomb repulsion between nuclei of equal charge, all atoms are ionised [3]:
2H+ + 3H+ → α (3.52 MeV) + 1n (14.06 MeV).
As can be seen, alpha particles and neutrons are the main products of this reaction. Through the latter, 80% of the energy of the D-T reaction is released, and since they are electrically neutral, they escape from magnetic confinement and can damage everything in their path, including the first wall and the fertile blanket. But, remember that the benefit of this reaction will be extracted from the kinetic energy (14.06 MeV) of this neutron flux. Therefore, the vessel wall must be able to optimally transfer and channel this kinetic energy to generate electricity following the proven thermal power plant scheme. The remaining 20 % of the energy emitted by the D-T reaction is via α-particles and other particles, which can also cause undesirable effects (erosion, blistering, etc.) on the plasma coating materials.
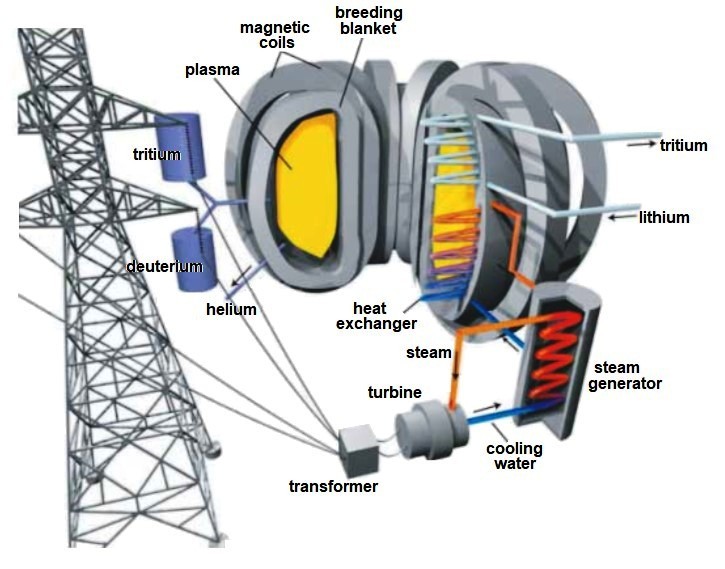
Neutron damage needs to be minimised
[3] Recall that an alpha particle, α, is a helium nucleus, 4He2+.
The neutron has a mass almost identical to that of the proton, but, unlike the proton, the neutron is electrically neutral and therefore does not have to pass through a Coulomb barrier. Thus, a neutron can interact vigorously with the atoms with which it collides, causing different kinds of damage to the structure of materials:
- Some, due to inelastic collisions, can produce transmutations (transforming one chemical element into a different one) that generate He, H and other impurities.
- Others, through elastic collisions, displace atoms, causing crystalline defects by pulling them out of their lattice positions. Components of future commercial fusion reactors must be able to withstand several tens of dpa [damage due to material degradation caused by neutrons is often quantified in terms of “displacements per atom” (dpa)].
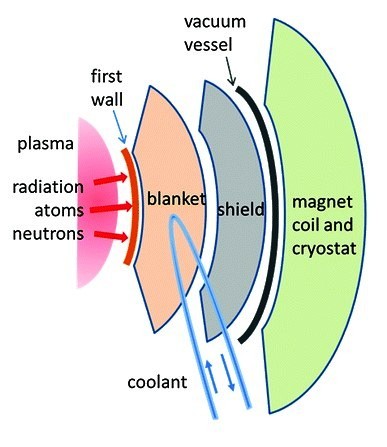
Materials in contact with an artificial Sun
Let us stress that safety is one of the distinctive peculiarities of the fusion science and technology community. Certainly, future reactors will be environmentally safe machines. To be so the materials for their construction have to be properly chosen on the basis of their properties, not only mechanical in the harsh environment of first-generation reactors, but also radiological (activity, decay heat, radiotoxicity) and, of course, with regard to their compatibility with other materials and cooling systems.
There is no doubt that a fusion plasma, at temperatures above 150 MK, is a difficult beast to tame. Various complex tools are used to soothe it, not only to control it, but also to efficiently transfer the energy produced and to remove impurities arising during the D-T reaction, which would also hinder it. This brings us to the so-called plasma facing materials. In addition to protecting the vessel from the hot plasma particles, they must actively transfer the enormous energy fluxes.
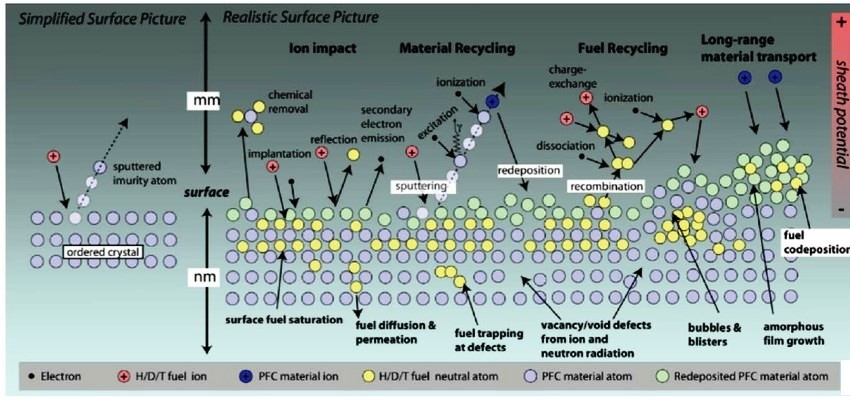
Low activation materials
The neutrons emitted by the D-T reaction, as mentioned above, are capable of causing transmutation, transforming stable atoms into radioactive ones. Thus, for fusion power generation to be an environmentally clean process, the materials used must be carefully chosen to prevent the production of long-lived radioactive waste.
From this perspective, in pursuit of the desired properties, the most satisfactory elements are Be, C, Cr, Fe, Si, Ta, Ti, V and W, on the basis of which low activation ferritic-martensitic steels (e.g. EUROFER97 and F82H steels) are being developed. These steels, developed to be able to work at temperatures up to 900 K, in addition to having quite adequate thermophysical properties and a remarkable resistance to swelling and embrittlement at high temperatures, show great compatibility with water or He-based coolants and Li-players (using a protective layer).
Difficult heat management (and heat transfer)
However, low neutron activation is not the only requirement that plasma cladding materials must satisfy. Recall that, coming from the plasma, there is a continuous and enormous heat flux of up to 20 MW/m² (for comparison, the flux of surface radiation on the Sun, given by the Stefan-Boltzmann equation, is 63.2 MW/m²). And this flux has to be managed.
To preserve the structural integrity of the material under such loads and to transfer such heat fluxes efficiently, two attributes become valuable: melting point and thermal conductivity, both of which must be high. Tungsten, with the highest melting point of all metals (3,695.2 K) and a thermal conductivity (170 W·m-1·K-1) eight times that of conventional steel, fortunately meets the low activation requirement. Tungsten, then, would seem to be the perfect candidate to cope with the harsh operating conditions in the first wall of a fusion reactor. But, as is often the case, nothing is perfect. Tungsten has two obvious drawbacks. On the one hand, its high atomic number means that just a negligible amount of tungsten is enough to cause the plasma to cool abruptly and shut down when contaminated. On the other hand, tungsten is very fragile. This means that, in addition to great difficulties in machining, the thermo-mechanical stresses that exist can easily cause cracks.
Let’s look at carbon. It is also an element with a low activation heat and a fairly high melting point (in fact, it does not melt, but sublimes directly at a temperature of 3,915 K). Moreover, because of its lower atomic number, even if it contaminates the plasma, it poses less danger of quenching it. Carbon fibre-reinforced SiC-SiC ceramic composites have therefore been studied with interest for plasma-facing (they also have high mechanical strength at temperatures around 1,300 K and good compatibility with He). Unfortunately, a number of disadvantages have also been discovered for carbon. One of the worst, apart from its ability to trap tritium (which rules it out for use in the first wall), is that, even when subjected to small doses of neutron irradiation (as low as 0.2 dpa), achievable in just two days of reactor operation, its thermal conductivity decreases by more than 80%. As a result, the material soon overheats, as the incident heat flux cannot be dissipated efficiently enough [1]. In contrast, under comparable conditions of neutron irradiation, tungsten hardly loses its thermal conductivity. SiC also shows significant amorphization and machinability problems.
The today and tomorrow of fusion materials. The IFMIF-DONES project
First generation fusion reactors are harsh working environments that require materials that do not collapse and that protect the integrity of the machine. Of the various alternatives currently under consideration (ferritic-martensitic steels, W and Ti alloys and SiC-SiC ceramic composites), ferritic-martensitic steels are the most technologically mature. However, in ITER the leading material will be 316-stainless steel, which is well suited to withstand the relatively low temperatures and pressures of an experimental reactor that will operate in intermittent campaigns. But in the DEMO project (whose objective, as we know, is to build a reactor which will be the first to inject electricity into the grid, confirming that fusion can be a commercially competitive energy source) the neutron flux will be continuous and much more intense, requiring ferritic-martensitic steels, much more robust material (the horizon of use of the other alternatives is still distant). In addition, the radiological aspects, not very relevant in the post-ITER case, are decisive for the commercial power industry, which must absorb their cost during the operation and subsequent decommissioning of the plant.
Consequently, for fusion to be economically viable, much effort still needs to be invested in the study of materials. Let us hasten to say that developing such materials is a challenge at the frontier of scientific and technological knowledge, many of whose refinements are the result of research at the plasma-wall interface, which is one of the main areas of work. However, in order to understand not only the nature of these materials and how their mechanical properties vary over the reactor lifetime, but also to qualify their suitability for their use in reactor components, IFMIF and, later, the IFMIF-DONES project were born. As we have seen in this series of articles, the IFMIF-DONES facility, which will provide neutron fluxes with intensity and energy similar to those in fusion reactors, is the only way in the near future that will allow researchers to examine how different materials hold up under real fusion conditions.
We are moving forward.
[4] It is possible that in the not-too-near future, second- or third-generation fusion reactors will not be based on the D-T reaction but on other reactions without neutron irradiation (which, as we know, require significantly higher temperatures for plasma ignition). In such aneutronic fusion reactors, carbon-based compounds could play a major role in the plasma cladding.